The time has come to pull renal cancer’s sweet tooth
The metabolic theory of cancer is based on cancer’s unique suppression of mitochondrial glucose oxidation and upregulation of cytosolic glycolysis, first identified by Otto Warburg nearly 100 years ago (1). This “Warburg effect” has been shown to be driven by numerous mechanisms including growth factor signalling, oncogene expression, metabolic enzyme mutation and regulation of factors that control mitochondrial homeostasis (2). Central to this metabolic remodelling is the inhibition of the mitochondrial pyruvate dehydrogenase complex (PDC), which is the key gate-keeping enzyme linking glycolysis to mitochondrial glucose oxidation. PDC converts the end-product of glycolysis, pyruvate, into acetyl-CoA which is then fed into the Krebs’ cycle in mitochondria to complete glucose oxidation (Figure 1A) (3). PDC activity is decreased by an inhibitory phosphorylation in Serine 293 by the mitochondrial enzyme pyruvate dehydrogenase kinase (PDK) although an additional inhibitory tyrosine phosphorylation by tyrosine kinases may contribute (2). Additional reasons resulting in decreased PDH activity include acetylation of the protein, for example by loss of the mitochondrial deacetylase Sirtuin3 (Sirt3); or by a decrease in mitochondrial calcium levels, which can result by the loss of a mitochondrial calcium transporter, i.e., the mitochondrial uncoupling protein 2 (UCP2) (4).
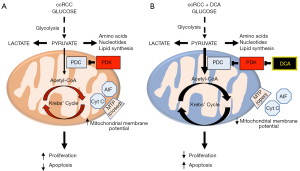
The Warburg effect revisited
Since the description of the Warburg effect, the suppression of mitochondrial glucose oxidation was thought to be irreversible and a “consequence” of the cancerous transformation of cells, perhaps a result of oxidative damage of the mitochondria. This meant that this secondary effect could not be a meaningful therapeutic target. In 2007 we published a paper showing for the first time that in several cancers the PDC inhibition and the suppression of mitochondrial oxidation were reversible (5) (Figure 1B). Pharmacologic inhibition of PDK with the small molecule dichloroacetate (DCA) resulted in increase in PDC activity and regression of tumors in vitro and in vivo (5). This suggested that PDC inhibition and suppression of mitochondrial glucose oxidation may be contributing to the cause of cancer. Indeed, there may be several advantages for the cancer cells that would undergo this metabolic remodelling (3,6) (Figure 1A).
First, the cells may now use the pyruvate that is not oxidized into the Krebs’ cycle, for biomass generation of amino acids (for example glutamine), nucleotides and lipids, through transamination and the pentose phosphate pathway (7). Second, the pyruvate may now be processed by functional nuclear PDC (mitochondrial PDC, but not its inhibitory PDK, can be translocated to the nucleus in cancer cells) to produce acetyl-CoA used to acetylate core histones involved in cell proliferation (8,9). Third, reduction in Krebs’ cycle metabolites, such as alpha-ketoglutarate, which acts as a cofactor for degradation of hypoxia inducible factors (HIF), creates an environment of pseudohypoxia, driving pro-angiogenic signaling (10). Fourth, the decreased supply of the Krebs’-derived electron donors (NADH and FADH2) to the electron transport chain, causes a hyperpolarization of the mitochondrial membrane potential, decreasing the open probability of the voltage-gated mitochondrial transition pore (MTP) and preventing the release of pro-apoptotic factors from the mitochondrial matrix, like cytochrome c and apoptosis inducing factor (AIF) (3,5). Overall, suppression of mitochondrial glucose oxidation increases cancer cell proliferation and angiogenesis while enhancing apoptosis resistance.
Yet, little is known about the importance of this metabolic remodeling in human tumors in vivo. In fact, there is evidence for variability in the degree of this mitochondrial remodelling. For example, in vivo studies have suggested that some lung and brain tumors may actually maintain a higher level of glucose oxidation than a pure “Warburg effect” would predict (11,12).
Clear cell renal cell cancer (ccRCC): a perfect example of the Warburg effect
A loss-of-function of the von Hippel Lindau (VHL) tumor suppressor is present in >90% of sporadic ccRCC tumors (13). This gene encodes a E3-ubiquitin ligase that degrades HIFs under normoxia. Thus its loss of function leads to the accumulation of HIF1α and HIF2α in normoxia, which are transcription factors that induce the expression of many pro-angiogenic factors like VEGF. Indeed, ccRCCs are heavily vascularised. But the attempts to inhibit these factors clinically have mostly failed, perhaps due to the multiplicity and overlapping nature of this downstream pro-angiogenic effect.
But HIFs also strongly induce the expression of PDK as well, thereby inhibiting PDC and mitochondrial glucose oxidation (14,15). Since HIFs also induce the expression of many glycolytic enzymes and glucose transporters, the combination of suppressed mitochondrial function and enhanced glycolysis would produce a strong Warburg effect. While this has been elucidated in vitro and in animal models [including ccRCC (16)], it has not been studied in ccRCC patients in vivo. Furthermore, FDG-PET imaging (the best available test to show an increase in glucose uptake because of enhanced glycolysis), while it shows increased glucose uptake in most solid tumors, it is difficult to interpret in ccRCC because FDG is present in the urine within the kidneys.
In a recent publication in Cell Metabolism, Courtney et al. elegantly demonstrated suppressed mitochondrial glucose oxidation in patient ccRCC tumors in vivo (17). In order to show this, the authors administered 13C-labelled glucose intravenously into five patients undergoing radical or partial nephrectomy for ccRCC. By using this “gold standard” method of in vivo metabolic studies, one can trace the fate of the labelled carbon atoms (using NMR and Mass Spectroscopy) through the enzymatic reactions in the glycolytic and Krebs’ cycle pathways. Immediately post-operatively, both tumor and adjacent normal kidney tissues were collected from the patients and metabolites were extracted and measured using NMR and Mass Spectrometry. The authors found an increase in 13C-labelled glycolytic intermediates including pyruvate and lactate as well as a reduction in 13C-labelled acetyl-CoA and all Krebs’ cycle metabolites as well as glutamine, in ccRCC compared to normal kidney tissues. This demonstrated a clear and strong inhibition of PDC activity resulting in increased glycolysis and decreased Krebs’ cycle activity.
Importantly, the authors adjusted the levels of the metabolites to the levels of glucose retained in the blood, since this shows some variability and is not clear whether 13C-glucose can reach steady state levels in the tumor tissue during the short intra-operative time. In summary, these data showed for the first time that human ccRCC tumors in vivo exhibit a strong Warburg effect, clearly distinguishing them from neighbouring non-cancer tissues. This suggests once again that this metabolic remodelling can provide many targets for the development of selective anti-cancer therapies. Since PDC activity inhibition is clearly demonstrated to be central to this, it begs the question whether reversal of this inhibition would be beneficial in humans with ccRCC. As discussed earlier, a single drug increasing PDC activity has the potential for multiple beneficial effects, decreasing both proliferation and apoptosis resistance within the ccRCC, without much effect in the non-cancerous tissues, which do not exhibit PDK induction. The fact that HIF is robustly activated in ccRCC (perhaps much more than in other solid tumors) given the loss of VHL, may make ccRCC an ideal target for PDK inhibitors.
Therapeutic targeting of suppressed PDC activity in ccRCC
We have previously shown that PDK is indeed strongly upregulated in human ccRCC tumors compared to normal adjacent kidney tissues (16) (Figure 2A). Following 13C-Glucose administration the the culture media of ccRCC cells DCA, by activating PDC, increased 13C-pyruvate-labeled conversion into 13C-acetyl-CoA, decreased pyruvate and lactate and increased Krebs’ metabolite levels; it also effectively increased mitochondrial respiration (Figure 2B). In a rodent xenotransplant model of human ccRCC, DCA effectively inhibited PDK, thus decreasing phosphorylation and increasing PDC activity (16). More importantly, DCA decreased proliferation, increased apoptosis, decreased vascularity and decreased tumor size after oral delivery in the animals (16) (Figure 2C). These data show that the Warburg effect described by Courtney et al. in human tumors (17), can be pharmacologically reversed.
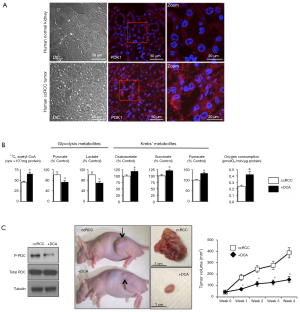
DCA has been used for the past 50 years as a treatment in patients with congenital mitochondrial deficiencies, including congenital PDC deficiency (18). After showing its potential efficacy as a cancer treatment in animals in 20075, we conducted a small early-phase trial with DCA in patients with advanced glioblastoma (19). In this study we obtained tumor tissue before and after DCA therapy and showed that once again, PDK was upregulated and inhibited by DCA, resulting in increased PDC activity and increased apoptosis within the tumor in vivo (19). While some patients showed evidence of tumor regression, the study size was too small to draw conclusions on overall efficacy but it clearly showed feasibility. The only known toxicity of the drug (i.e., a dose-dependent and reversible peripheral neuropathy) was mostly absent in the doses used. Similar results in feasibility and safety were shown in subsequent early-phase trials with DCA in cancer patients (20-22) as well as in a trial of DCA in patients with Pulmonary Arterial Hypertension (4). This is a proliferative vascular disease in which the proliferating smooth muscle cells in pulmonary arteries of affected patients exhibit a Warburg effect and have a cancer-like pro-proliferative and anti-apoptotic phenotype. While a variability in response was shown with DCA in both cancer and PAH patients, this later PAH study showed that this variable response to DCA may depend on genetic factors. Loss-of-function polymorphisms in Sirt3 and UCP2 that would result in PDK-independent inhibition of PDC, were associated with resistance to DCA (4). Perhaps such polymorphisms (that would result in inhibition of many mitochondrial enzymes, not just PDC) may determine the exact nature of the metabolic remodeling in tumors as well as their potential response to DCA. Data obtained in vivo with a similar methodology to Courtney et al. (17) showed that some human lung and brain cancer patients exhibit a less prominent Warburg effect (with some persistence of glucose oxidation) compared to ccRCC (11,12). Again, this maybe because the strong induction of PDK in ccRCC may be more effective in inhibiting PDC than in other tumors. It also means that because of this, ccRCC may be, among many other solid tumors, the ideal cancer to treat with DCA.
We believe the time is right to consider clinical trials of DCA in patients with ccRCC. DCA is generic (and thus cheap) but this also suggests the initiation of such trials by academic investigators, as industry will not be interested. On the other hand, there are plenty of data available that have found a safe and potentially effective dose of the drug. Importantly, the nice methodology provided by Courtney et al., may allow proof for the ability of DCA to reverse the PDC inhibition in vivo by comparing the metabolic profile in the excised tumors in ccRCC patients pre-treated with DCA. Combined with data on potential presence of Sirt3 and UCP2 polymorphisms in ccRCC patients [that may identify DCA-resistant patients (4)], this will be an attractive precision medicine approach in ccRCC.
Lastly, there is currently a lot of interest in studying the role of immune cells infiltrating ccRCC tumors and several check-point inhibitors are currently clinically tested. Although the role of PDC activity or the effects of DCA have not been directly tested in immune cells, it cannot escape our attention that it is now well-proven that a significant suppression in glucose oxidation and upregulation of glycolysis (compatible with PDC inhibition and a Warburg effect) is an early and necessary event in the process of T cell activation (23). Thus it is possible that a similar metabolic remodeling in infiltrating immune cells could have contributed to the metabolic profile of the whole tumor tissue shown by Courtney et al. (17). It is also possible that DCA may have additional beneficial effects in ccRCC perhaps combined with check-point inhibitors.
Diagnostic implications of the ccRCC Warburg effect
Another potential clinical implication of the Courtney et al. work may be in the diagnostic approach to kidney cancer. One of the major barriers to diagnosing ccRCC is the misclassification of benign oncocytomas as ccRCC. Neither CT, nor MRI or PET imaging have been shown to be effective in differentiating the two lesions. This inability to radiographically differentiate between oncocytoma and ccRCC leads to surgical extirpation of a benign mass in up to 15–20% of patients (24). Histologically, oncocytomas are characterized by a very large increase in the number of mitochondria compared to normal kidney and ccRCC. While the function of these mitochondria has not been systematically studied, it is very unlikely that they would exhibit PDC inhibition, because they do not carry VHL mutations and thus there is no reason to expect HIF upregulation and PDK induction. Thus it is possible that measurement of metabolites similar to the ones measured in tumor tissue ex vivo by Courtney et al. (17), by in vivo magnetic resonance spectroscopy, compared to levels seen in adjacent tissues, would separate the two tumors based on the presence of a “Warburg effect” or not. Early evidence of the feasibility approach in human brain in vivo is available but more work will be needed to establish its feasibility in human kidney tumors.
Conclusions
While the sample size of the patients studied by Courtney et al. is small (n=5), this study has potentially significant clinical implications, both diagnostically and therapeutically for the management of ccRCC patients. Combined with preclinical data and experience with metabolic modulators like DCA in human cancer and vascular disease, this work strengthens the rationale to inhibit the Warburg effect (and its multiple pro-tumor effects) in ccRCC by pulling its sweet tooth, i.e., the inhibited PDC.
Acknowledgments
Funding: None.
Footnote
Provenance and Peer Review: This article was commissioned and reviewed by the Section Editor Peng Zhang, MD, PhD (Department of Urology, Zhongnan Hospital of Wuhan University, Wuhan, China).
Conflicts of Interest: Both authors have completed the ICMJE uniform disclosure form (available at http://dx.doi.org/10.21037/tcr.2018.12.31). The authors have no conflicts of interest to declare.
Ethical Statement: The authors are accountable for all aspects of the work in ensuring that questions related to the accuracy or integrity of any part of the work are appropriately investigated and resolved.
Open Access Statement: This is an Open Access article distributed in accordance with the Creative Commons Attribution-NonCommercial-NoDerivs 4.0 International License (CC BY-NC-ND 4.0), which permits the non-commercial replication and distribution of the article with the strict proviso that no changes or edits are made and the original work is properly cited (including links to both the formal publication through the relevant DOI and the license). See: https://creativecommons.org/licenses/by-nc-nd/4.0/.
References
- Warburg O. Metabolism of tumours. Biochem Zeitschr 1923;142:317-33.
- Kinnaird A, Michelakis ED. Metabolic modulation of cancer: a new frontier with great translational potential. J Mol Med (Berl) 2015;93:127-42. [Crossref] [PubMed]
- Sutendra G, Michelakis ED. Pyruvate dehydrogenase kinase as a novel therapeutic target in oncology. Front Oncol 2013;3:38. [Crossref] [PubMed]
- Michelakis ED, Gurtu V, Webster L, et al. Inhibition of pyruvate dehydrogenase kinase improves pulmonary arterial hypertension in genetically susceptible patients. Sci Transl Med 2017;9: [Crossref] [PubMed]
- Bonnet S, Archer SL, Allalunis-Turner J, et al. A mitochondria-K+ channel axis is suppressed in cancer and its normalization promotes apoptosis and inhibits cancer growth. Cancer Cell 2007;11:37-51. [Crossref] [PubMed]
- Hanahan D, Weinberg RA. Hallmarks of cancer: the next generation. Cell 2011;144:646-74. [Crossref] [PubMed]
- Vander Heiden MG, Cantley LC, Thompson CB. Understanding the Warburg effect: the metabolic requirements of cell proliferation. Science 2009;324:1029-33. [Crossref] [PubMed]
- Sutendra G, Kinnaird A, Dromparis P, et al. A nuclear pyruvate dehydrogenase complex is important for the generation of acetyl-CoA and histone acetylation. Cell 2014;158:84-97. [Crossref] [PubMed]
- Kinnaird A, Zhao S, Wellen KE, et al. Metabolic control of epigenetics in cancer. Nat Rev Cancer 2016;16:694-707. [Crossref] [PubMed]
- Sutendra G, Dromparis P, Kinnaird A, et al. Mitochondrial activation by inhibition of PDKII suppresses HIF1a signaling and angiogenesis in cancer. Oncogene 2013;32:1638-50. [Crossref] [PubMed]
- Hensley CT, Faubert B, Yuan Q, et al. Metabolic Heterogeneity in Human Lung Tumors. Cell 2016;164:681-94. [Crossref] [PubMed]
- Maher EA, Marin-Valencia I, Bachoo RM, et al. Metabolism of [U-13 C]glucose in human brain tumors in vivo. NMR Biomed 2012;25:1234-44. [Crossref] [PubMed]
- Moore LE, Nickerson ML, Brennan P, et al. Von Hippel-Lindau (VHL) inactivation in sporadic clear cell renal cancer: associations with germline VHL polymorphisms and etiologic risk factors. PLoS Genet 2011;7:e1002312. [Crossref] [PubMed]
- Kim JW, Tchernyshyov I, Semenza GL, et al. HIF-1-mediated expression of pyruvate dehydrogenase kinase: a metabolic switch required for cellular adaptation to hypoxia. Cell Metab 2006;3:177-85. [Crossref] [PubMed]
- Keith B, Johnson RS, Simon MC. HIF1alpha and HIF2alpha: sibling rivalry in hypoxic tumour growth and progression. Nat Rev Cancer 2011;12:9-22. [Crossref] [PubMed]
- Kinnaird A, Dromparis P, Saleme B, et al. Metabolic Modulation of Clear-cell Renal Cell Carcinoma with Dichloroacetate, an Inhibitor of Pyruvate Dehydrogenase Kinase. Eur Urol 2016;69:734-44. [Crossref] [PubMed]
- Courtney KD, Bezwada D, Mashimo T, et al. Isotope Tracing of Human Clear Cell Renal Cell Carcinomas Demonstrates Suppressed Glucose Oxidation In Vivo. Cell Metab 2018;28:793-800.e2. [Crossref] [PubMed]
- Stacpoole PW, Kurtz TL, Han Z, et al. Role of dichloroacetate in the treatment of genetic mitochondrial diseases. Adv Drug Deliv Rev 2008;60:1478-87. [Crossref] [PubMed]
- Michelakis ED, Sutendra G, Dromparis P, et al. Metabolic modulation of glioblastoma with dichloroacetate. Sci Transl Med 2010;2:31ra34. [Crossref] [PubMed]
- Chu QS, Sangha R, Spratlin J, et al. A phase I open-labeled, single-arm, dose-escalation, study of dichloroacetate (DCA) in patients with advanced solid tumors. Invest New Drugs 2015;33:603-10. [Crossref] [PubMed]
- Dunbar EM, Coats BS, Shroads AL, et al. Phase 1 trial of dichloroacetate (DCA) in adults with recurrent malignant brain tumors. Invest New Drugs 2014;32:452-64. [Crossref] [PubMed]
- Garon EB, Christofk HR, Hosmer W, et al. Dichloroacetate should be considered with platinum-based chemotherapy in hypoxic tumors rather than as a single agent in advanced non-small cell lung cancer. J Cancer Res Clin Oncol 2014;140:443-52. [Crossref] [PubMed]
- Leavy O. T cells: Mitochondria and T cell activation. Nat Rev Immunol 2013;13:224. [Crossref] [PubMed]
- Kutikov A, Fossett LK, Ramchandani P, et al. Incidence of benign pathologic findings at partial nephrectomy for solitary renal mass presumed to be renal cell carcinoma on preoperative imaging. Urology 2006;68:737-40. [Crossref] [PubMed]