Proton radiotherapy in the treatment of lung cancer
Introduction
Ever since the first attempts to treat malignancy with radiotherapy were made in the early 1900s, delivering a tumorcidal dose of radiotherapy while minimizing toxicity to nearby normal tissues has always been a challenge. Initially, tumors could be targeted only via direct or near-direct contact with a radiotherapy source. With the advent of Cobalt-60 radiation sources and, later, linear accelerators, therapeutic radiation could be delivered to virtually any site in the body. However, the dose that can be delivered to the tumor continues to be limited by normal tissue constraints. Fundamentally, this is determined by the physical characteristics of standard photon or electron radiotherapy. Photons, which include standard X-rays, and electrons deposit the radiation dose over the entire track of the beam; after peaking at a physically determined depth in water (or tissue), the deposited dose decreases slowly. For example, as shown in Figure 1, the maximum dose of radiation delivered by a standard 6 MV photon beam is at a depth of 1.5 cm in water. For electrons of similar energies, the depth at which maximum dose is delivered (Dmax) is even less. This dose distribution is reasonable for superficial tumors, but for tumors more than 1.5 cm below the surface of the skin, for one radiation beam, the normal tissue proximal to the tumor will be treated to a higher dose than the tumor itself. This physical reality is compensated for in standard radiotherapy by the use of multiple beams that converge at the level of the tumor. With more advanced planning techniques, such as intensity-modulated radiotherapy (IMRT), the intensity of each beam can be altered by using a computer-determined “best solution” for all beams to maximize tumor dose while sparing surrounding normal tissue. Despite these significant advances, standard radiotherapy continues to be limited by the generally inalterable physical characteristics of a photon (or electron) beam. This has led to interest in other forms of radiotherapy with different beam characteristics. Here we focus primarily on proton radiotherapy, the most common charged particle therapy in clinical use for lung cancer in the United States.
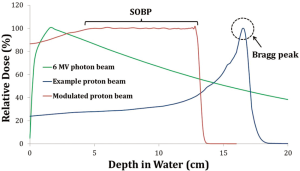
Physical characteristics of proton beams
As previously stated, photon beams reach their maximum dose (Dmax) at a known depth in tissue, a known physical property determined by the beam energy. Higher photon energies lead to greater Dmax at the expense of increased fall-off dose as well as increased possibility of neuton scattering. However, charged particles such as protons have minimal ionization along their beam path, meaning that the dose delivered to any point along the beam path is minimal and the entrance dose for any particular proton beam is less than that for a comparable photon beam. Instead, the vast majority of dose in a charged particle beam is deposited near the end of the beam path, when the particles have nearly stopped (Figure 1). This phenomenon was initially observed as early as 1904, and has been dubbed the “Bragg peak” for its discoverer William Henry Bragg (1). By modulating the proton energy, the depth of the Bragg peak, or point of maximal radiation dose delivery, can be altered. However, the area of the Bragg peak for any one proton energy is too narrow for clinical use, requiring the use of a summed proton beam of multiple energies, resulting in the so-called “spread-out Bragg peak” (Figure 1).
A concept useful in comparing forms of radiotherapy is that of relative biologic effectiveness (RBE). Simply stated, this is a ratio between a standard dose of radiation (typically 250 kVp X-rays) and the dose of the test radiation required to produce the same biologic effect. Although the concept is fairly simple, derivation of the RBE is a complex process, depending upon a number of variables including the type of tissue being studied, the degree of hypoxia within the tissue, the type of radiation being used, the dose delivered, and the energy lost over the beam path (linear energy transfer or LET). Historically, the RBE for a variety of different types of radiation has been determined primarily by in vitro and preclinical studies. For clinical use, the RBE for a proton beam (within the Bragg peak) is generally assumed to be 1.1 (2,3), meaning that for every 1 Grey (Gy), the biological effectiveness of a proton beam is similar to what is seen with 1.1 Gy of standard X-rays. This has led to the use of the term cobalt-Grey equivalent (CGE) when describing doses or proton therapy. Thus 74 CGE is equivalent to 67.3 Gy delivered by protons. Although the RBE/CGE concept provides a clinically useful value, several caveats must be borne in mind. The RBE is thought to vary slightly over the breadth of a Bragg peak. Specifically, the experimentally determined RBE values within a proton beam generally increase over the Bragg peak and are highest in the final millimeters (4-8). This effect is recognized in the course of routine clinical treatments by the recommendation that no proton beam should terminate in a critical normal structure. RBE also varies as a function of the tissue irradiated; in vivo preclinical models have predicted average values ranging from 0.7 to 1.6 (2). Examination of this variation in the RBE of a proton beam has led to attempts to integrate this factor into treatment planning (9-11).
Clinical use of proton therapy in lung cancer
The use of proton radiotherapy has grown substantially, particularly over the past decade, with 10 facilities using this modality in the United States alone. The unique characteristics of proton radiotherapy has led to its use being championed to allow both sparing of normal tissue and increasing the radiation dose delivered to targets heretofore limited by proximity to adjacent normal surrounding structures. Particular interest in proton radiotherapy has been expressed for the treatment of lung cancer. The standard therapy for locally advanced lung cancer involves a combination of radiation and chemotherapy delivered concurrently, typically to radiation doses of 60-70 Gy. However, treatment in this dose range can be quite toxic, leading to significant pulmonary injury (mainly pneumonitis and fibrosis) as well as esophagitis and other toxic effects (12). Any damage to the lungs in patients with lung cancer tends to be exacerbated by a lack of pulmonary reserve, as many patients present with some form of chronic obstructive disease from cigarette smoking and many require supplemental oxygen even before radiotherapy. The findings regarding the value of dose-escalation in these patients is somewhat conflicting (13,14). One possibility for this disparity could be treatment-related toxicity; in other words, although tumor control may be increased in patients treated with higher radiation doses, the commensurate increase in toxicity and toxicity-related death can mask any potential benefit. Hence the desire for a radiation treatment modality that can minimize radiation dose to critical structures (e.g., the lungs) while allowing the possibility of dose escalation to the target. One such modality that attempts to achieve this goal is proton beam therapy.
Several planning studies have been done to compare the dose to normal surrounding structures associated with either photon or proton radiotherapy. Generally, in these studies, proton beam therapy has shown benefits over standard conformal radiotherapy; specifically, the dose to the uninvolved lung can in some cases be superior to that provided via conventional radiotherapy (15-17) or IMRT (15,17). Examples of a typical plan for passive scatter proton radiotherapy and one for IMRT are shown in Figure 2. Proton radiotherapy may also have advantages over photon-based stereotactic radiotherapy for smaller tumors in terms of sparing normal tissue (15,18-20). However, the benefit from the use of protons from the dosimetric perspective is not universal. Because of the uncertainty of the exact range of the Bragg peak, particularly in hypodense tissues such as lung, the use of additional margin of high-dose radiation may be required, leading to a higher dose to critical structures, particularly when they are close to the target (21). Further, because many tumors have irregular borders and involve the mediastinum, highly conformal IMRT may provide an advantage in regard to normal tissue sparing compared with the traditional passive-scatter approach to proton radiotherapy (22).
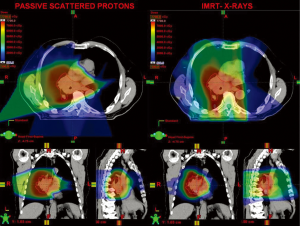
Dosimetric studies aside, a growing body of literature details the clinical experience of using charged particle therapy for lung cancer. Several institutions have generated significant data from the use of proton radiotherapy as monotherapy. One of the earliest published studies reported the investigators’ experience in treating mainly early-stage non-small cell lung cancer (NSCLC) with a proton boost after traditional photon radiotherapy. In that study of 37 patients, the local control rate was 87%, and only 2 patients developed symptomatic pneumonitis (23). Studies of stereotactic or hypofractionated proton-based radiotherapy for early-stage lung cancer have shown similar local control rates for small, peripheral lesions (24-27). However, in the same studies, local control rates for larger lesions have been less favorable, falling in the range of 40% to 60%. Toxicity in these studies has been minimal; in a phase I/II trial recently completed at MD Anderson Cancer Center involving a dose of 87.5 CGE, the rates of symptomatic pneumonitis and esophagitis were 11% and 6% (27).
Less information is available regarding combinations of proton radiotherapy with concurrent chemotherapy for locally advanced lung cancer. The guiding principle for radiotherapy to the lung has been to increase the dose to the point of maximum tolerability, as a radiation dose-response relationship has been observed for locally advanced lung cancer (13). However, any dose escalation must take into account the significant toxicity associated with thoracic radiotherapy. In fact, the most recent national trial of dose-escalated thoracic radiotherapy initiated by the RTOG led to the premature closure of the high-dose treatment group (74 Gy) because of the absence of any observed survival benefit (14). Although the final toxicity data from this trial were not available when this review was written, it is worth noting that 7 patients died in the high-dose group versus 3 in the control group (treated to 60 Gy). Thus, it seems that significant caution should be observed in attempting dose escalation of thoracic radiotherapy when that therapy involves conventional methods. However, a recent retrospective review of concurrent platinum-based chemotherapy and proton radiotherapy noted particularly low rates of pneumonitis (2%) and esophagitis (5%) compared with those rates in a similar group of patients treated to a lower dose (63 Gy) by either 3-dimensional conformal radiotherapy or IMRT (28). Further investigation of these results in a phase II trial showed similarly positive results, with local control rates of close to 80% and pneumonitis and esophagitis rates of around 2% and 11% (29). These results are also being evaluated further in a Bayesian randomized trial of image-guided proton radiotherapy compared with photon radiotherapy for patients with locally advanced lung cancer.
Despite the dosimetric evidence and some clinical data supporting the use of proton radiotherapy for the treatment of lung cancer, significant challenges remain. First, treatment planning using proton radiotherapy is complicated by the inherent motion of the lung. Unlike photon radiotherapy, protons are drastically affected by the material through which they pass. Thus tissue densities must be accounted for during treatment planning. However the motion of the lung - and consequently the motion of the tumor - during the respiratory cycle can make this challenging, particularly in light of the finite range of protons. Although proton radiotherapy is appealing in the context of sparing normal structures, any changes in the path of the beams during respiration could change the range of the proton beam significantly, leading to marginal misses of the target or increased dose to surrounding normal structures. This problem has been addressed in several planning studies [reviewed in (30)], and a variety of different approaches are being used to minimize this problem. In one such approach, “smearing” the target volume artificially increases the volume targeted in an attempt to ensure good coverage despite small changes arising from motion during the respiratory cycle. This problem of appropriate targeting is further amplified by changes in the tumor itself during radiotherapy: tumors can shrink or become more cavitary in response to radiotherapy, which again changes the density of the tissue traversed by the proton beam and altering its range. At MD Anderson Cancer Center, we have tried to minimize this problem by obtaining images throughout the course of the radiotherapy and modifying the plans (“adaptive planning”) if the tumor responds significantly.
Further difficulties arise from highly irregular targets. As noted previously, IMRT can in many cases provide more conformal treatment for large irregular lesions. In an initial dosimetric comparison between IMRT and proton radiotherapy as part of the above-mentioned randomized protocol, IMRT was found to have a dosimetric advantage in many cases (31). One possible solution to the problem of conformality is the use of some form of modulated proton radiotherapy. Conventional proton radiotherapy (“passive scatter”) uses material to scatter the beam over a large area, with a rotating wheel placed in the beam path to allow generation of a spread-out Bragg peak. This approach basically delivers a uniform dose over the extent of the target, but does not allow generation of irregular contours for the dose to be delivered. The concept of “pencil beam” proton radiotherapy is being investigated to improve upon this dose distribution; in this technique, the dose can be “painted” over any particular target by the use of pencil beams of protons directed at small segments of an individual target. Although this approach can improve the conformal coverage of irregular targets, in some situations it can be less robust than use of a passive scatter beam, because the accuracy of scanning beam proton radiotherapy is affected to an even greater extent by organ motion (32). Studies of the use of scanning beam technology for the treatment of lung cancer are ongoing.
Finally, cautions have been raised regarding the problem of unintended neutron dose when using proton radiotherapy. The production of secondary neutrons may be of particular concern for passive scatter beams, in which a physical component is placed in the beam path, because these scattered neutrons may themselves be carcinogenic. Although this could be of significant import for younger populations, patients with lung cancer tend to be older, and risk estimates for carcinogenesis are highest for young patients (33). Further, the magnitude of neutrons generated by passive scatter beams is debated in the literature (34). Regardless, the advent of scanning beam technology has greatly reduced the possible risk of neutron scatter in the use of proton beam radiotherapy (33).
Future challenges and opportunities
The advent of proton radiotherapy for lung cancer brings with it an opportunity to minimize the toxicity of current standard-of-care therapy. At present, this possibility is being investigated in at least one randomized trial in which the benefits of passive scatter proton radiotherapy are being compared with those of IMRT. Further prospective studies are also ongoing to compare the benefits of stereotactic proton radiotherapy with those of standard 3D-conformal stereotactic radiotherapy. So, what further challenges and opportunities remain? One major criticism of proton radiotherapy for the treatment of lung cancer is its cost. The development of proton radiotherapy capability requires a significant cost outlay for any institution. Moreover, a treatment course of proton radiotherapy is significantly more expensive at the current time than is a comparable course of IMRT. Although this cost will likely decrease with time, proton radiotherapy remains an expensive treatment option. However, the costs of proton radiotherapy for lung cancer must be weighed against the costs of toxicity associated with therapy. In fact, in one cost-effectiveness model involving only recent studies, proton radiotherapy was found to be cost-effective for the management of selected cases of lung cancer (35). This finding underscores the idea that merely calculating treatment costs does not completely measure the value of any particular therapy.
With regard to technology, the current “cutting edge” in proton radiotherapy delivery is the development of intensity modulation. As noted previously, one of the disadvantages of passive beam proton radiotherapy is the inability to conform to a highly irregular target or to allow dose-painting within the irradiated field. Scanning beam technology removes this disadvantage. Moreover, one could conceivably generate treatment plans that take advantage of the increased RBE at the Bragg peak by deliberately encompassing a radioresistant area of a tumor (e.g., an hypoxic area) within the Bragg peak of each scanning beam. Theoretically, this approach would lead to improved response without incurring any toxicity associated with dose escalation. However, as noted previously, scanning beam technology for proton delivery is highly dependent on precise planning software and improved motion management. As these technologies improve, true intensity modulation of protons in the treatment of lung cancer will become a reality.
In summary, the current state of proton beam radiotherapy or intensity-modulated proton beam radiotherapy for lung cancer is one of optimism. Prospective trials of proton radiotherapy are ongoing, and those findings, as they mature, will be valuable in further clarifying the role of proton radiotherapy for the management of this deadly disease.
Acknowledgments
Funding: None.
Footnote
Provenance and Peer Review: This article was commissioned by the Guest Editors (Huan Giap and Eric Y Chuang) for the series “Particle Beam Therapy II” published in Translational Cancer Research. The article has undergone external peer review.
Conflicts of Interest: Both authors have completed the ICMJE uniform disclosure form (available at http://dx.doi.org/10.3978/j.issn.2218-676X.2012.12.02). The series “Particle Beam Therapy II” was commissioned by the editorial office without any funding or sponsorship. The authors have no other conflicts of interest to declare.
Ethical Statement: The authors are accountable for all aspects of the work in ensuring that questions related to the accuracy or integrity of any part of the work are appropriately investigated and resolved.
Open Access Statement: This is an Open Access article distributed in accordance with the Creative Commons Attribution-NonCommercial-NoDerivs 4.0 International License (CC BY-NC-ND 4.0), which permits the non-commercial replication and distribution of the article with the strict proviso that no changes or edits are made and the original work is properly cited (including links to both the formal publication through the relevant DOI and the license). See: https://creativecommons.org/licenses/by-nc-nd/4.0/.
References
- Bragg W. On the ionization of various gasses by the alpha particles of radium. Proc Phys Soc 1907;20:523-50.
- Paganetti H, Niemierko A, Ancukiewicz M, et al. Relative biological effectiveness (RBE) values for proton beam therapy. Int J Radiat Oncol Biol Phys 2002;53:407-21. [PubMed]
- International Commission on Radiation Units and Measurements. Prescribing, recording, and reporting proton-beam therapy (ICRU report 78). Bethesda, MD.
- Wouters BG, Lam GK, Oelfke U, et al. Measurements of relative biological effectiveness of the 70 MeV proton beam at TRIUMF using Chinese hamster V79 cells and the high-precision cell sorter assay. Radiat Res 1996;146:159-70. [PubMed]
- Bettega D, Calzolari P, Chauvel P, et al. Radiobiological studies on the 65 MeV therapeutic proton beam at Nice using human tumour cells. Int J Radiat Biol 2000;76:1297-303. [PubMed]
- Tang JT, Inoue T, Inoue T, et al. Comparison of radiobiological effective depths in 65-MeV modulated proton beams. Br J Cancer 1997;76:220-5. [PubMed]
- Courdi A, Brassart N, Hérault J, et al. The depth-dependent radiation response of human melanoma cells exposed to 65 MeV protons. Br J Radiol 1994;67:800-4. [PubMed]
- Matsuura T, Egashira Y, Nishio T, et al. Apparent absence of a proton beam dose rate effect and possible differences in RBE between Bragg peak and plateau. Med Phys 2010;37:5376-81. [PubMed]
- Frese MC, Wilkens JJ, Huber PE, et al. Application of constant vs. variable relative biological effectiveness in treatment planning of intensity-modulated proton therapy. Int J Radiat Oncol Biol Phys 2011;79:80-8. [PubMed]
- Carabe A, Moteabbed M, Depauw N, et al. Range uncertainty in proton therapy due to variable biological effectiveness. Phys Med Biol 2012;57:1159-72. [PubMed]
- Jones B, Underwood TS, Dale RG. The potential impact of relative biological effectiveness uncertainty on charged particle treatment prescriptions. Br J Radiol 2011;84:S61-9. [PubMed]
- O’Rourke N, Roqué I. Concurrent chemoradiotherapy in non-small cell lung cancer. Cochrane Database Syst Rev 2010;CD002140 [PubMed]
- Partridge M, Ramos M, Sardaro A, et al. Dose escalation for non-small cell lung cancer: analysis and modelling of published literature. Radiother Oncol 2011;99:6-11. [PubMed]
- Bradley JD, Paulus R, Komaki R, et al. A randomized phase III comparison of standard-dose (60 Gy) versus high-dose (74 Gy) conformal chemoradiotherapy +/− cetuximab for stage IIIa/IIIb non-small cell lung cancer: Preliminary findings on radiation dose in RTOG 0617. Int J Radiat Oncol Biol Phys 2011;81: abstr LBA2.
- Chang JY, Zhang X, Wang X, et al. Significant reduction of normal tissue dose by proton radiotherapy compared with three-dimensional conformal or intensity-modulated radiation therapy in Stage I or Stage III non-small-cell lung cancer. Int J Radiat Oncol Biol Phys 2006;65:1087-96. [PubMed]
- Lee CH, Tait D, Nahum AE, et al. Comparison of proton therapy and conformal X-ray therapy in non-small cell lung cancer (NSCLC). Br J Radiol 1999;72:1078-84. [PubMed]
- Nichols RC, Huh SN, Henderson RH, et al. Proton radiation therapy offers reduced normal lung and bone marrow exposure for patients receiving dose-escalated radiation therapy for unresectable stage iii non-small-cell lung cancer: a dosimetric study. Clin Lung Cancer 2011;12:252-7. [PubMed]
- Macdonald OK, Kruse JJ, Miller JM, et al. Proton beam radiotherapy versus three-dimensional conformal stereotactic body radiotherapy in primary peripheral, early-stage non-small-cell lung carcinoma: a comparative dosimetric analysis. Int J Radiat Oncol Biol Phys 2009;75:950-8. [PubMed]
- Hoppe BS, Huh S, Flampouri S, et al. Double-scattered proton-based stereotactic body radiotherapy for stage I lung cancer: a dosimetric comparison with photon-based stereotactic body radiotherapy. Radiother Oncol 2010;97:425-30. [PubMed]
- Wang C, Nakayama H, Sugahara S, et al. Comparisons of dose-volume histograms for proton-beam versus 3-D conformal x-ray therapy in patients with stage I non-small cell lung cancer. Strahlenther Onkol 2009;185:231-4. [PubMed]
- Seco J, Panahandeh HR, Westover K, et al. Treatment of non-small cell lung cancer patients with proton beam-based stereotactic body radiotherapy: dosimetric comparison with photon plans highlights importance of range uncertainty. Int J Radiat Oncol Biol Phys 2012;83:354-61. [PubMed]
- Zhang X, Li Y, Pan X, et al. Intensity-modulated proton therapy reduces the dose to normal tissue compared with intensity-modulated radiation therapy or passive scattering proton therapy and enables individualized radical radiotherapy for extensive stage IIIB non-small-cell lung cancer: a virtual clinical study. Int J Radiat Oncol Biol Phys 2010;77:357-66. [PubMed]
- Bush DA, Slater JD, Bonnet R, et al. Proton-beam radiotherapy for early-stage lung cancer. Chest 1999;116:1313-9. [PubMed]
- Bush DA, Slater JD, Shin BB, et al. Hypofractionated proton beam radiotherapy for stage I lung cancer. Chest 2004;126:1198-203. [PubMed]
- Iwata H, Murakami M, Demizu Y, et al. High-dose proton therapy and carbon-ion therapy for stage I nonsmall cell lung cancer. Cancer 2010;116:2476-85. [PubMed]
- Nihei K, Ogino T, Ishikura S, et al. High-dose proton beam therapy for Stage I non-small-cell lung cancer. Int J Radiat Oncol Biol Phys 2006;65:107-11. [PubMed]
- Chang JY, Komaki R, Wen HY, et al. Toxicity and patterns of failure of adaptive/ablative proton therapy for early-stage, medically inoperable non-small cell lung cancer. Int J Radiat Oncol Biol Phys 2011;80:1350-7. [PubMed]
- Sejpal S, Komaki R, Tsao A, et al. Early findings on toxicity of proton beam therapy with concurrent chemotherapy for nonsmall cell lung cancer. Cancer 2011;117:3004-13. [PubMed]
- Chang JY, Komaki R, Lu C, et al. Phase 2 study of high-dose proton therapy with concurrent chemotherapy for unresectable stage III nonsmall cell lung cancer. Cancer 2011;117:4707-13. [PubMed]
- Liao Z, Lin SH, Cox JD. Status of particle therapy for lung cancer. Acta Oncol 2011;50:745-56. [PubMed]
- Mohan R, Zhang X, Matney J, et al. IMRT vs. passively scattered proton therapy (PSPT) for locally advanced non-small cell lung cancer (LA NSCLC) randomized trial - is there equipoise? Int J Radiat Oncol Biol Phys 2010;78:S201-2.
- Schippers JM, Lomax AJ. Emerging technologies in proton therapy. Acta Oncol 2011;50:838-50. [PubMed]
- Brenner DJ, Hall EJ. Secondary neutrons in clinical proton radiotherapy: a charged issue. Radiother Oncol 2008;86:165-70. [PubMed]
- Paganetti H, Bortfeld T, Delaney TF. Neutron dose in proton radiation therapy: in regard to Eric J. Hall (Int J Radiat Oncol Biol Phys 2006;65:1-7). Int J Radiat Oncol Biol Phys 2006;66:1594-5; author reply 1595. [PubMed]
- Grutters JP, Pijls-Johannesma M, Ruysscher DD, et al. The cost-effectiveness of particle therapy in non-small cell lung cancer: exploring decision uncertainty and areas for future research. Cancer Treat Rev 2010;36:468-76. [PubMed]